Translating CRISPR Gene-Editing into a Groundbreaking Cell Therapy
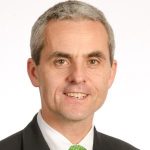
Bill Lundberg, CEO, Merus; former CSO, CRISPR Therapeutics
A little more than 10 years have passed since the discovery of CRISPR gene editing. It’s been a whirlwind development for science and medicine. We are now on the cusp of seeing the first medicine based on this powerful technology win approval from regulators in the US and Europe.
It’s exagamglogene autotemcel (exa-cel), a cell therapy developed by CRISPR Therapeutics and in partnership with Vertex Pharmaceuticals. The treatment is made when a patient’s blood is withdrawn, hematopoietic stem cells are isolated in the lab, they are gene-edited to produce high levels of fetal hemoglobin, and then the edited cells are re-infused back into the patient.
These engineered cells, after a single shot, have been shown to successfully produce enough fetal hemoglobin-expressing and oxygen-carrying red blood cells to effectively cure patients with sickle-cell disease and transfusion-dependent beta-thalassemia. Vertex and CRISPR Therapeutics have completed their application for FDA approval.
This is a landmark achievement for the biotech history books. It’s both a meaningful treatment for patients with no good options, and it’s a testimony to our industry’s capacity to turn basic science into life-changing medicines.
I was there in the early days as the first US-based employee and chief scientific officer of CRISPR Therapeutics. Let me tell you a bit about how this medicine came to be, along with some lessons that we learned along the way.
Getting Started
We opened our first lab in Boston in 2015. Within three years, we filed to begin our first clinical trials in Europe for CTX-001, now called exa-cel. CRISPR Therapeutics raised a $25 million Series A financing from Versant Ventures in April 2014. One year later, we raised a $29 million Series B financing from that included Vertex, SR One, Celgene, and Bayer.
We had resources, but not unlimited resources. We had to think about where to start with a technology that could potentially change any gene in the genome, or could potentially treat any one of hundreds of different diseases.
The first big decision was about where to concentrate our valuable time and energy. We couldn’t afford to be distracted, or risk spreading ourselves too thin.
We chose initially to pursue sickle cell disease and transfusion-dependent beta-thalassemia. This was a logical place to start.
Every patient with sickle cell disease has the exact same molecular change. Every patient has the same clinical manifestations of rigid, sticky, and misshapen red blood cells that can clump together and damage the lining of blood vessels, and organs. Every patient suffers from terrible pain crises that end up requiring emergency medical care. Every patient has respiratory problems. Fatigue is a constant battle. Patients suffer terribly for years, and they die too soon.
We knew that while patients endure a variety of symptoms, we had a chance to take them all out if we could deliver a single, elegant edit to those blood-forming stem cells. (The related disease beta-thalassemia, with similar rationale and evaluation of therapeutic approaches is not discussed further here).
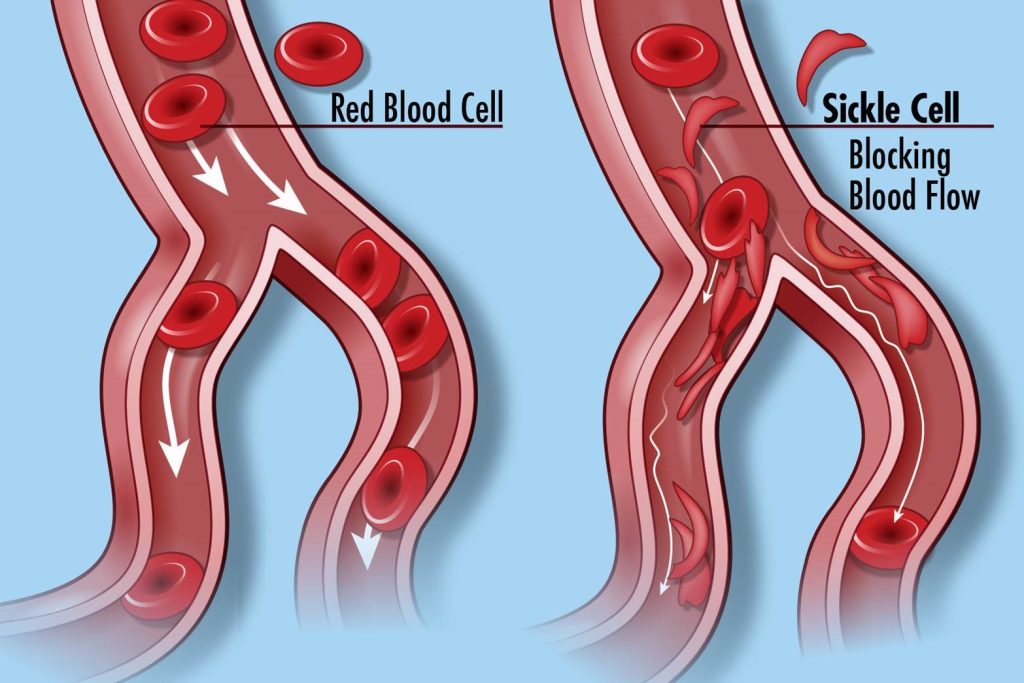
By National Human Genome Research Institute (NHGRI) Creative Commons license.
We also knew that if we went down this path, we could find out quickly in the lab if we were wrong. Biomarkers of disease and treatment efficacy – both short-term and long-term – could be easily obtained from a blood draw. And we wouldn’t have to wait for a long-term follow-up study to tell us if the biological edit caused an improvement in the clinical outcomes that matter most.
The genetic defect, as alluded to above, is in the hematopoietic system, from which cells can be removed, studied, manipulated (gene edited), and returned to the patient. Several gene therapy companies that came before us already established processes that were well-understood. Perhaps equally important, we knew that sickle cell disease was severe enough that doctors and patients would consider trying out a brand-new technology to treat it, even though that naturally meant taking on some unknown risks.
Once settling on sickle cell disease and beta-thalassemia as our top priorities, we then had to think as clearly as possible about the questions to ask. The first and most obvious one was:
“What target should we edit?”
One option was to correct the genetic defect itself in the beta-globin gene, by using CRISPR to “knock-in” the correct code. However, we found early on that such a gene correction approach was less efficient than other approaches described below, and we were concerned we may not be able to correct enough of the cells to treat the disease. The CRISPR edits also cause gene disruption, which might knock out beta-globin function and cause thalassemia major, making the patients worse.
This strategy wasn’t worth the risk, and we dropped it quickly.
Before spending too much time and money in the lab, it helped a great deal to work with experts in the field and dig deeply into the literature. We went back to re-read a seminal finding published back in 1948, by Janet Watson, a pediatrician in New York. She observed that infants with sickle cell disease hadn’t yet developed symptoms and proposed that this was related to the protective effects of a fetal form of hemoglobin in the blood at birth.
Remarkably, the infants Watson studied only developed symptoms 6-9 months after birth, when the adult beta globin gene, or the sickle globin variant in patients, was fully expressed. In 1955, scientists found that some adult patients had only mild symptoms. These patients were no longer infants, but they appeared to have a persistent expression of an “alkali resistant” form of hemoglobin known as fetal hemoglobin, which was responsible for ameliorating symptoms. Later studies confirmed that increased levels of fetal hemoglobin is associated with reduced clinical manifestations, and acute pain episodes.
These observations decades ago made it clear: If scientists could spur more expression of fetal hemoglobin in adults, either through gene therapy or gene editing, it could make a big impact against the disease. There are now many reported inherited variations in gene sequences that are known to cause long-lasting expression of fetal hemoglobin into adulthood. Perhaps re-creating one of these rare variants in cells from a patient could ameliorate disease. Importantly, prior work by others had validated this approach, including in mouse models here and here.
Another approach was suggested by genome-wide association studies here, here and here that identified more commonly occurring variations in different genes that had more modest effects. These led to a series of remarkable discoveries, essential for our later work: the BCL11A gene is a master regulator of fetal hemoglobin. Removing it alone — a single factor — from the red blood cell compartment leads to high fetal hemoglobin and cures sickle cell disease in a mouse model; and ultimately identifying the critical sequences in the BCL11A gene that controls its function in the red blood cell compartment, called an erythroid enhancer.
Perhaps we could aim our CRISPR gene editing efforts at BCL11A or other targets identified by genome wide studies, to cause high levels of fetal hemoglobin and cure sickle cell disease. At CRISPR Therapeutics, we extensively evaluated in the laboratory these two approaches: re-creating variants associated with hereditary persistence, and disrupting sequences identified by the genome wide association studies and subsequent BCL11A studies. We were also guided by important concurrent work from multiple academic laboratories also pursuing similar approaches, for example here, here and here.
As we evaluated different potential approaches, we needed to keep asking ourselves the most pertinent questions.
“How much gene editing is needed?”
We intended to edit the hematopoietic stem cell, which gives rise to the disease-causing red blood cells. The ‘edited’ hematopoietic stem cell contains the genetic code to produce both the fetal hemoglobin that would be protective, and the defective sickle hemoglobin that is the source of so many problems.
But here’s where we were fortunate: fetal hemoglobin is extremely potent in overcoming the toxic effects of sickle hemoglobin. As little as 25-30% fetal hemoglobin appears protective, slowing down by 10,000-fold the sickling process of hemoglobin polymerization in the low oxygen state that causes symptoms. That provides ample time for the red blood cell to circulate back to the oxygen-rich lungs, to prevent the onset of sickling.
We were also fortunate that not all red blood cells in a patient had to be corrected to reduce or prevent the worst symptoms – the vaso-occlusive crises that often send patients to the Emergency Department. Only two-thirds of the red blood cells in a patient need to be of the healthy and non-sickling type to dramatically reduce the risk of stroke, as shown in studies of blood transfusion therapy.
We also realized that far less than two-thirds of the hematopoietic stem cells that give rise to red blood cells actually needed to be corrected, because these blood cells that are protected from sickling will last longer in circulation and accumulate over time. As little as 20-25% correction of the precursor hematopoietic stem cells could give rise to this important two-thirds blood cell threshold, based on computer models and experiments in mice.
Remarkably, these predictions matched the observations here, here and here from rare patients who carried a mix of both healthy donor cells from transplantation and also some of their original, disease-associated cells.
This was an important signal to us. The bar wasn’t impossibly high.
Once we settled on our strategy for gene editing, and we had an idea of the threshold we needed to clear, we had to ask ourselves the same question over and over in different ways —
“Did we achieve sufficient editing?”
We presented our initial results at the American Society of Hematology annual meetings in 2016 and 2017, where we demonstrated 80% editing efficiency of the hematopoietic stem cells – far greater than the 25% needed. The process we invented delivered greater than 30% fetal hemoglobin expression. These values met or exceeded our goals, and, together with other foundational work from academia supporting our approach, encouraged us to move forward into the clinic with the lead candidate CTX-001 (now exa-cel), which uses CRISPR gene editing to disrupt the erythroid enhancer of the BCL11A gene.
It can be easy to get immersed in the details of the biology or new technology or only focus on the efficacy measures. But we had to remain rooted in the most basic question of all —
“Will it be safe?”
The body produces an estimated 2-3 million red blood cells per second. With the nature of our edit, it had to be safe, precise, and it had to persist. We would only find out the answers to these questions in clinical trials.
We wondered why the intended change we were making with our gene editing had never been observed in a patient, despite the evolution of hundreds of other naturally occurring genetic variations that both increased fetal hemoglobin in patients and reduced their symptoms. Might our particular edit have some other negative effects that we had not yet uncovered? We knew that loss of BCL11A function outside of the red cell compartment had other negative effects, shown here and here.
In contrast, our approach with CTX-001 limits the BCL11A disruption specifically to the red blood cell lineage. We didn’t have direct validation from human genetics to lean back on, to give us confidence that it would be safe. However, extensive foundational academic work — for example here and here — and our own preclinical experiments were critical in providing further support for our approach.
Early on, concerns had been raised in the scientific community about the potential for ‘off-target’ effects elsewhere in the genome with CRISPR gene editing. At ASH 2017, we addressed this concern directly. The specific guide RNA (site for editing) we selected showed no evidence of off-target activity in a deep evaluation of thousands of potential off-target sites, and the edited cells still functioned normally.
Furthermore, there was no evidence of clonal (precancerous) growth and no evidence of cancer formation in the standard Good Laboratory Practice (GLP) toxicology studies. The cumulative body of evidence to that point supported our decision to go to the clinic with CTX-001.
From the start we were faced with the question:
“How do we industrialize this?”
When we began, it was unclear how best to apply this new CRISPR gene editing approach. Should the Cas9 nuclease component be delivered as protein or encoded in RNA or DNA? Should we use separate CRISPR and TRACR RNA fragments — as occurs naturally in bacteria — or use a concatenated single guide RNA? Should RNA be synthesized or transcribed? Or contain bases that are chemically modified? Introduced into the cell by transfection, viral transduction or electroporation? Which source was best for starting and in-process materials? How to establish GLP materials and methods? How to bring experimental-grade processes up to Good Manufacturing Practice (GMP) standards?
Our research and process development teams urgently evaluated different variables and suppliers to assess and determine the optimal approach; we relied, as much as possible, on well-established methods and providers for obtaining, managing, manipulating and evaluating hematopoietic stem cells; for process development and manufacturing; and iterating through variations at scale with GLP-like materials and under GMP-like conditions where necessary. This was about reducing as many variables as possible, over time, to keep focused on the immediate problem at hand: to invent a CRISPR gene editing process for patients that was effective, robust and reliable.
We also wondered:
“How will we address the ethical and regulatory challenges?”
Like other early entrants, we faced considerable scientific and public concern about the ethical and societal consequences of human gene editing. Much of the concern centered on heritable modifications to the germ line, in which changes could be passed on future generations, with no turning back.
This concern about “editing humanity” or “designer babies” led some to call for a total ban on human gene editing for therapeutic purposes. That would derail or delay not only our own progress and ability to finance our company, but also the efforts of the entire field. We all had to address it thoughtfully.
We engaged consultants and obtained an evaluation from the ethicists and clinicians at the Stanford Center for Biomedical Ethics. Together with another gene editing company, we met with the White House Office of Science and Technology Policy as well as members of Congress and staff on Capitol Hill to both educate and learn.
We participated in the first International Summit on Human Gene Editing at the National Academies of Sciences, Engineering and Medicine in December 2015. We published a peer-reviewed position paper recommending that CRISPR gene editing for therapeutic approaches should “treat the patient, not [modify] the germline.” We were hoping to build broad support for somatic (non-heritable) gene editing of the kind we were developing in CTX-001.
We did not know whether the regulatory landscape for CRISPR gene editing would be permissive. We relied on established FDA Guidance and EMA Guideline documents and precedents as much as possible. We engaged extensively with consultants and sought early advice from national health authorities. We formed partnerships with clinical trialists and clinicians with deep experience in genetics, pathophysiology, disease management, and transplantation medicine.
With this support, we developed clinical protocols that incorporated the appropriate patient selection, treatment plans, endpoints and evaluation, and biomarkers and thresholds for success. We worked hard to ensure that our clinical plans continued to be aligned with regulatory guidance, guidelines and precedent.
The rapid development of exa-cel, from opening our labs in 2015 to first clinical trial application in Europe in 2017, could only have been possible through the dedication, hard work and focus of the teams at CRISPR Therapeutics, with the help and support of our partner Vertex, the deep knowledge and expertise of our many collaborators, and the foundational understanding of red cell biology and disease pathogenesis built over the past 70 years, summarized here.
The impact on patients with sickle cell disease has been remarkable. As of Dec 2022, all 31 sickle cell disease patients treated with exa-cel remain free of transfusions or vaso-occlusive crises.
Rarely in our careers are we given the opportunity to bring a brand-new technology, in this case CRISPR gene editing, to an important and well-understood medical need. We are all grateful to be able to contribute to this landmark achievement, the first CRISPR gene editing medicine.
This article is adapted from a talk given at the 80th birthday symposium in honor of MIT professor Robert Weinberg.